Asynchronous muscles
Asynchronous muscles are muscles in which there is no one-to-one relationship between electrical stimulation and mechanical contraction. These muscles are found in 75% of flying insects and have convergently evolved 7-10 times.[1] Unlike their synchronous counterparts that contract once per neural signal, mechanical oscillations trigger force production in asynchronous muscles. Typically, the rate of mechanical contraction is an order of magnitude greater than electrical signals.[1] Although they achieve greater force output and higher efficiency at high frequencies, they have limited applications because of their dependence on mechanical stretch.
Structure
Molecular
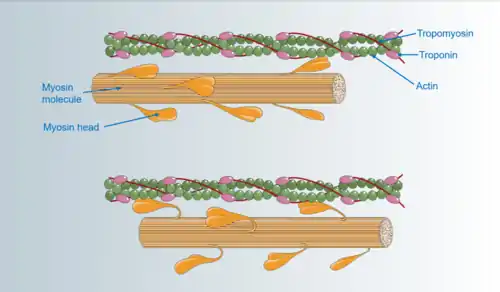
The exact molecular mechanisms used by asynchronous muscles are unknown, but it is believed that asynchronous muscles have no unique molecular structures as compared to their synchronous counterparts. A study investigating the asynchronous power muscles in bumblebees with X-ray diffraction videos showed that actin and myosin alone are sufficient for generating asynchronous behavior.[2] This finding helps explain how asynchronous muscles independently evolved across insect taxa.[1] More recent work using similar X-ray diffraction techniques in Lethocerus discovered that troponin bridges may play a critical role in stretch activation. As the muscle is stretched, these bridges move tropomyosin to reveal myosin-actin binding sites.[3] The muscle can only produce force when these sites are activated.
Macroscopic
Several changes to asynchronous muscles' macroscopic structure provide it with high force production and efficiency at high contraction frequencies. A critical adaptation is that asynchronous muscles maintain a tonic level of calcium instead of cycling calcium between contractions. This is evident in their long twitch duration. This is due to relatively spare sarcoplasmic reticulum. Because of requirements for high force production, myofiber and myofibril diameters are increased and the large amount of ATP necessary leads to high mitochondria densities.[1] In Cotinus mutabilis, asynchronous muscles are composed of 58.1% myofibril, 36.7% mitochondria, and 1.6% sarcoplasmic reticulum. In comparison, synchronous muscles in Schistocerca americana are composed of 65% myofibril, 23.5% mitochondria and 9.6% sarcoplasmic reticulum.[1] Although synchronous muscle has a higher percentage of myofibril, the cross-sectional area of asynchronous myofibril is 3.7 µm2 as opposed to 0.82 µm2 in synchronous muscle for the previously described species.[1]
Properties
Asynchrony between electrical stimulation and muscle contraction
The defining characteristic of asynchronous muscles is that there is no direct relationship between neural activation and muscle contraction. Typically, the number of muscle contractions is an order of magnitude greater than the number of action potentials sent to the muscle. Instead of directly controlling force generation, neural signals maintain [Ca2+] above a threshold for stretch-activation to occur.[4] For asynchronous muscles, neural inputs are typically thought of as an "on-off" switch while mechanical stimulus leads to individual muscle contractions. However, recent studies using genetically engineered Drosophila revealed correlations between [Ca2+] and force production.[5] Further work has shown bilateral calcium asymmetries in Drosophila.[4] These results indicate that there is some level of neural control beyond a simple "on" or "off" state.
Delayed stretch activation and delayed shortening deactivation
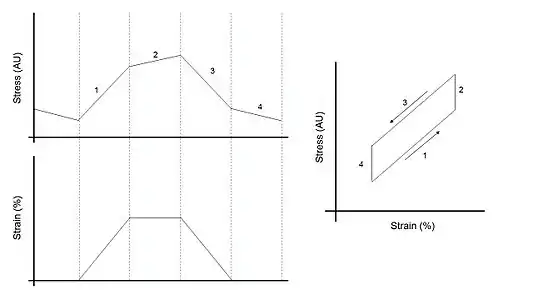
Delayed stretch activation and delayed shortening deactivation allow asynchronous muscles to generate positive work under cyclic oscillations.[6] When the muscle shortens, force drops and continues dropping even when the muscle length remains constant. Similarly, when the muscle lengthens, force increases and continues increasing after the muscle length remains constant.[1] Because of these delays, the work produced by the muscle during shortening is greater than the work absorbed during lengthening, therefore producing positive work. In contrast, synchronous muscles absorb work under similar conditions.[1] Both types of muscles consume ATP to drive force production and produce work.[6]
Long twitch duration
Long twitch duration is a functional consequence of the macroscopic properties of asynchronous muscle. Because asynchronous muscle can generate power without cycling calcium between contractions, the required rate of calcium regulation is significantly slower. In addition to the reduction in sarcoplasmic reticulum, relatively large myofibril diameters lead to increased diffusion times of Ca2+.Under isometric twitch experiments, asynchronous muscle in Cotinus mutabilis were found to have a twitch duration of 125 ms. In the same study, synchronous muscle in Schistocerca americana had a twitch duration of 40 ms.[1] Therefore, asynchronous muscles respond slowly to neural stimulus. In the case of insect flight, electrical stimulation alone is too slow for muscle control. For Cotinus mutabilis, the twitch duration is ten times as long as a wingbeat period.[1]
Functional significance
Resonant properties
Asynchronous muscles produce work when they undergo mechanical oscillations provided there is sufficient Ca2+.[1][6] This can be achieved in one of two ways. First, two antagonistic muscles can be configured with elastic structures such that the contraction of one muscle stretches the other, causing it to activate and vice versa. This configuration is found in the power muscles of flying insects.[7] Second, a single asynchronous muscle can deform an elastic element which then stretches the muscle and causes the muscle to contract again. This setup is used by Drosophila to oscillate mechanosensory organs known as halteres.[8] As long as neural stimulus turn the muscles "on," both systems will continue to oscillate. These systems can be thought of as resonant systems, for which the oscillation frequency is dependent on the elasticity, damping, and force applied to the system.[9]
In a simplified case, this can be thought of as a linearly damped harmonic oscillator, for which the damped resonant frequency is
The damping ratio, ζ , is dependent on c, the damping coefficient, m, the mass of the system, and k, the stiffness of the system as shown
Power-control tradeoffs
Asynchronous muscles sacrifice neural control and flexibility in exchange for high force production and efficiency. Given the long twitch duration of asynchronous muscle, neural control is too slow to power flight. For instance, the asynchronous muscles in Cotinus mutabilis contract ten times faster than expected given their twitch duration.[1] Because these muscles rely on stretch activation, they must be configured such that they can be stretched by an external force. Furthermore, they are only useful when evolutionary pressures select for a muscle that reactively contracts against an imposed stretch. For example, in grasping tasks, it would be detrimental for antagonist muscles to spontaneously contract. Despite these disadvantages, asynchronous muscles are beneficial for high frequency oscillations. They are more efficient than synchronous muscles because they do not require costly calcium regulation.[6] This allows for changes in their macroscopic structure for increased force production.
Applications

Insect flight
Miniaturization of insects leads to high wingbeat frequencies with midges reaching wingbeat frequencies of 1000 Hz.[10] Because of their high force production and efficiency, asynchronous muscles are used to power insect flight in 75% of species. These insects possess two pairs of antagonistic asynchronous muscles that produce the majority of the power required for flight. These muscles are oriented such that as one pair contracts, it deforms the thorax and stretches the other pair, causing the second pair to contract.[7] The same thoracic deformations oscillate the wings. By utilizing the elastic thorax to store and return energy during wing deceleration and subsequent acceleration, Drosophila is able to reduce energetic costs by 10%.[11] This leads to a highly-efficient resonant system.
When wingbeat frequencies match the resonant frequency of the muscle-thorax system, flight is most efficient. In order to change wingbeat frequencies to avoid obstacles or generate more lift, insects use smaller "control" muscles such as the pleurosternal muscles to stiffen the thorax.[9] From the equations in the Resonant properties section, it is clear that the natural frequency of the system increases with stiffness. Therefore, modulating the stiffness of the thorax leads to changes in wingbeat frequency.
Mammalian hearts
Although heart muscles are not strictly asynchronous, they exhibit delayed stretch activation properties. As cardiac muscle is lengthened, there is an instantaneous rise in force caused by elastic, spring-like elements in the muscle. After a time delay, the muscle generates a second rise in force, which is caused by delayed stretch activation as seen in purely asynchronous muscle.[12] This property benefits heart function by maintaining papillary muscle tension during the entire systolic cycle well after the electrical wave has passed.[12] Through stretch activation, the heart can rapidly adapt to changes in heart rates.
Bioinspired robotics
Because of challenges arising from miniaturization such as poor scaling of electric motors, researchers have turned towards insects to develop centimeter-scale flying robots.[13] Although the actuators in the RoboBee are not asynchronous, they use elastic elements to transmit forces from its "muscles" (piezoelectric actuators) to flap the wings. Similar to flying insects, they exploit resonance to improve efficiency by 50%.[14]
References
- Josephson, R. K.; Malamud, J. G.; Stokes, D. R. (2000-09-15). "Asynchronous muscle: a primer". Journal of Experimental Biology. 203 (18): 2713–2722. ISSN 0022-0949. PMID 10952872.
- Iwamoto, H.; Yagi, N. (2013-09-13). "The Molecular Trigger for High-Speed Wing Beats in a Bee". Science. 341 (6151): 1243–1246. doi:10.1126/science.1237266. ISSN 0036-8075. PMID 23970560. S2CID 32645102.
- Perz-Edwards, Robert J.; Irving, Thomas C.; Baumann, Bruce A. J.; Gore, David; Hutchinson, Daniel C.; Kržič, Uroš; Porter, Rebecca L.; Ward, Andrew B.; Reedy, Michael K. (2011-01-04). "X-ray diffraction evidence for myosin-troponin connections and tropomyosin movement during stretch activation of insect flight muscle". Proceedings of the National Academy of Sciences. 108 (1): 120–125. doi:10.1073/pnas.1014599107. ISSN 0027-8424. PMC 3017141. PMID 21148419.
- Wang, Qian; Zhao, Cuiping; Swank, Douglas M. (2011-11-02). "Calcium and Stretch Activation Modulate Power Generation in Drosophila Flight Muscle". Biophysical Journal. 101 (9): 2207–2213. doi:10.1016/j.bpj.2011.09.034. PMC 3207158. PMID 22067160.
- Gordon, Shefa; Dickinson, Michael H. (2006-03-14). "Role of calcium in the regulation of mechanical power in insect flight". Proceedings of the National Academy of Sciences of the United States of America. 103 (11): 4311–4315. doi:10.1073/pnas.0510109103. ISSN 0027-8424. PMC 1449689. PMID 16537527.
- Syme, D. A. (2002-08-01). "How to Build Fast Muscles: Synchronous and Asynchronous Designs". Integrative and Comparative Biology. 42 (4): 762–770. doi:10.1093/icb/42.4.762. ISSN 1540-7063. PMID 21708773.
- Boettiger, Edward G.; Furshpan, Edwin (1952-06-01). "The mechanics of flight movements in diptera". The Biological Bulletin. 102 (3): 200–211. doi:10.2307/1538368. ISSN 0006-3185. JSTOR 1538368.
- Deora, Tanvi; Singh, Amit Kumar; Sane, Sanjay P. (2015-02-03). "Biomechanical basis of wing and haltere coordination in flies". Proceedings of the National Academy of Sciences. 112 (5): 1481–1486. doi:10.1073/pnas.1412279112. ISSN 0027-8424. PMC 4321282. PMID 25605915.
- Dickinson, Michael H; Tu, Michael S (1997-03-01). "The Function of Dipteran Flight Muscle". Comparative Biochemistry and Physiology Part A: Physiology. 116 (3): 223–238. doi:10.1016/S0300-9629(96)00162-4.
- Sotavalta, Olavi (1953-06-01). "Recordings of high wing-stroke and thoracic vibration frequency in some midges". The Biological Bulletin. 104 (3): 439–444. doi:10.2307/1538496. ISSN 0006-3185. JSTOR 1538496.
- "Muscle efficiency and elastic storage in the flight motor of Drosophila - ProQuest". search.proquest.com. Retrieved 2017-04-30.
- Vemuri, Ramesh; Lankford, Edward B.; Poetter, Karl; Hassanzadeh, Shahin; Takeda, Kazuyo; Yu, Zu-Xi; Ferrans, Victor J.; Epstein, Neal D. (1999-02-02). "The stretch-activation response may be critical to the proper functioning of the mammalian heart". Proceedings of the National Academy of Sciences. 96 (3): 1048–1053. doi:10.1073/pnas.96.3.1048. ISSN 0027-8424. PMC 15348. PMID 9927691.
- Wood, R. J. (2008-04-01). "The First Takeoff of a Biologically Inspired At-Scale Robotic Insect". IEEE Transactions on Robotics. 24 (2): 341–347. doi:10.1109/TRO.2008.916997. ISSN 1552-3098. S2CID 6710733.
- Jafferis, N. T.; Graule, M. A.; Wood, R. J. (2016-05-01). Non-linear resonance modeling and system design improvements for underactuated flapping-wing vehicles. 2016 IEEE International Conference on Robotics and Automation (ICRA). pp. 3234–3241. doi:10.1109/ICRA.2016.7487493. ISBN 978-1-4673-8026-3. S2CID 15100511.