Ocean storage of carbon dioxide
Ocean storage of carbon dioxide (CO2) is a method of carbon sequestration. The concept of storing carbon dioxide in the ocean was first proposed by Italian physicist Cesare Marchetti in his 1976 paper "On Geoengineering and the carbon dioxide problem."[1] Since then, the concept of sequestering atmospheric carbon dioxide in the world's oceans has been investigated by scientists, engineers, and environmental activists. 39,000 GtC (gigatonnes of carbon) currently reside in the oceans while only 750 GtC are in the atmosphere.[2][3]
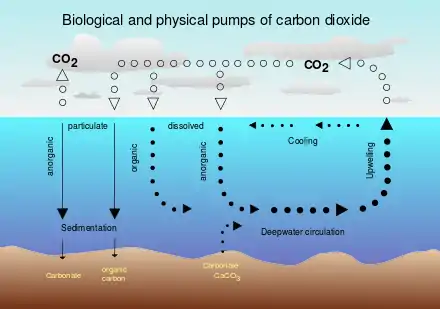
Part of a series on the |
Carbon cycle |
---|
![]() |
Of the 1300 Gt carbon dioxide from anthropogenic emissions over the last 200 years, about 38% of that has already gone into the oceans.[2] Carbon dioxide is currently emitted at 10 GtC per year and the oceans currently absorb 2.4 Gt carbon dioxide per year. The ocean is an enormous carbon sink with the capacity to hold thousands more gigatons of carbon dioxide. Ocean sequestration has the potential to decrease atmospheric carbon dioxide concentrations according to some scientists.
Ocean chemistry

After atmospheric carbon dioxide dissolves into the ocean, the aqueous carbon dioxide reacts with seawater to form carbonic acid.[4] As carbonic acid continues to interact with water molecules, carbonate is formed, which increases the concentration of hydrogen ions in the ocean and consequently reduces ocean pH. Therefore, increasing carbon dioxide concentrations in the atmosphere decreases ocean pH.[5] However, calcium carbonate acts as a buffer to large decreases in pH. As pH decreases, calcium carbonate dissolution increases.[6] The IPCC uses 0.1 pH as a marker for acceptable change in ocean pH comparable to natural pH fluctuations without noticeable environmental effects. With a 0.1 pH change, the IPCC estimates that 1000 GT of carbon could be stored in the ocean and decrease negative environmental effects if the same quantity of carbon remained in the atmosphere.[5] If carbon dioxide remained in the atmosphere, the resulting change in ocean pH would also be similar as ocean mixing would eventually absorb excess carbon dioxide.[5]
Dilute carbon dioxide injection and storage
Dilute carbon dioxide injection requires injection at depths where carbon dioxide can be dispersed by ocean currents and ocean mixing. Upon injection, waters interact and mix based on density and dilute the concentration of carbon dioxide.[5] Boat based carbon dioxide injection distributes low carbon dioxide concentrations in open waters while moving to increase carbon dioxide dispersal area. carbon dioxide dispersal via boat can also occur by a pipe attached to the ship injecting a dilute carbon dioxide mixture into the water column. The carbon dioxide is usually injected at 1000 m depth to reduce carbon dioxide bubble escape. As injected carbon dioxide bubbles rise, dispersal increases up the water column.[7] Large dispersal areas and low concentrations of carbon dioxide significantly decrease any significant changes in local pH and resulting effects on marine ecosystems.[7] Wickett et al. used measurements in natural pH fluctuations from atmospheric carbon dioxide to suggest that dilute carbon dioxide injection at 0.37 GTC/ yr would have a negligible effect on ocean pH.[5] Dilute ocean injection requires little infrastructure in comparison to other forms of ocean injection.[4] The IEA Greenhouse Gas R&D Programme estimates that dilute carbon dioxide injection would cost $70 per tonne of carbon dioxide including costs of carbon capture, transport, and storage before boat dispersal.[8]
Release of solid carbon dioxide at depth
Carbon dioxide ocean storage can occur through solid or solid hydrate of carbon dioxide. The solid state density of carbon dioxide is approximately 1.5 times greater than seawater and thus tends to sink to the ocean floor. The dissolving rate at the surface is about 0.2 cm/hr such that a small quantity of carbon dioxide can be completely dissolved before reaching the sea floor.[5] In addition to solid carbon dioxide injection, carbon dioxide hydrate is another popular method for storage. Hydrate formation takes place when the dissolved concentration of liquid carbon dioxide is around 30% and 400 meters below sea level. Hydrates form as an external layer around liquid carbon dioxide droplets or as a solid mass.[9] The molecular composition is composed of carbon dioxide and water, carbon dioxide•nH2O (n ≈ 5.75).[4] The resulting density is denser than seawater by approximately 10%. Compared to liquid carbon dioxide, the hydrate form dissolves significantly slower in seawater, at about 0.2 cm/hr as well.[9] Additionally, the hydrate remains immobile on the seafloor and forms hydrate cap, forcing liquid carbon dioxide to only move laterally.[10] The overall molecular stability relies on the temperature and pressure of the environment, and hydrates only dissociate when placed in direct contact with additional heat and water at concentrations below its equilibrium concentration.[11] However, due to its crystalline structure, pure hydrate doesn't travel through pipes. Given that 100% efficiency is extremely difficult to achieve, in reality, both laboratory and field experiments suggest that sinking reaction efficiency is approximately 15-25%.[4] Any kind of instability of hydrates is likely to cause dissolution and dispersion during the descending or injection process.[10]
Mineralization and deep sea sediments
Similar to mineralization processes that takes place within rocks, mineralization can also occur under the sea. The rate of dissolution of carbon dioxide from atmosphere to oceanic regions relies upon the circulation period of the ocean and buffering ability of subducting surface water.[11] Researches have demonstrated that the carbon dioxide marine storage at several kilometers deep could be viable for up to 500 years, but is dependent on injection site and conditions. Several studies have shown that although it may fix carbon dioxide effect, carbon dioxide may be released back to the atmosphere over time. However, this is unlikely for at least a few more centuries. The neutralization of CaCO3, or balancing the concentration of CaCO3 on the seafloor, land and in the ocean, can be measured on a timescale of thousands of years. More specifically, the predicted time is 1700 years for ocean and approximately 5000 to 6000 years for land.[12][13] Further, the dissolution time for CaCo3 can be improved by injecting near or downstream of the storage site.[14]
In addition to carbon mineralization, another proposal is deep sea sediment injection. It injects liquid carbon at least 3000 m below the surface directly into ocean sediments to generate carbon dioxide hydrate. Two regions are defined for exploration: the negative buoyancy zone (NBZ), which is the region between liquid carbon dioxide denser than surrounding water and where liquid carbon dioxide has neutral buoyancy, and hydrate formation zone (HFZ), which typically has low temperatures and high pressures. Several research models have shown that the optimal depth of injection requires consideration of intrinsic permeability and any changes in liquid carbon dioxide permeability for optimal storage. The formation of hydrates decreases liquid carbon dioxide permeability, and injection below HFZ is more energetically favored than within the HFZ. If the NBZ is a greater column of water than the HFZ, the injection should happen below the HFZ and directly to the NBZ. In this case, liquid carbon dioxide will sink to the NBZ and be stored below the buoyancy and hydrate cap. Carbon dioxide leakage can occur if there is dissolution into pore fluid or via molecular diffusion. However, this occurs over thousands of years.[14][15][16]
Carbon dioxide plumes
Researchers have been able to show in lab and in small in situ experiments that carbon dioxide can be injected into oceans as rising or sinking plumes.[5] The plume will sink if it is more dense than seawater. This should occur if the plume, a mix of carbon dioxide and seawater, is injected at depths of 3 km.[4] As the plume moves vertically, it will dissolve at least partially due to convective mass transfer with the passing seawater. Dissolution is increased by increased currents perpendicular to the vertical water column containing the plume because of the increase convective mass transfer. For sinking plumes, minimal horizontal currents are desired so that the plume can sink to the ocean floor for longer term sequestration. The opposite is desired for rising plumes, which similarly to other previously mentioned dilute ocean storage techniques, relies on the dispersal to make the change in carbon dioxide concentration in the ocean low enough to not affect the marine biosphere significantly.[17]
The proposed method of injection is droplets of supercritical carbon dioxide mixed with seawater. Plumes can be engineered to dissolve at different rates based on the size, concentration, and rate of injection of the carbon dioxide/seawater droplets. For rising plumes which rely on dissolution to sequester carbon dioxide, a smaller droplet with a greater rate of injection is better because it leads to faster dissolution. Sinking plumes ideally form lakes of carbon dioxide on the ocean floor for more long term sequestration.[5]
Carbon dioxide lakes
Carbon dioxide lakes will form on ocean floors in depressions or trenches in the seabed. These lakes sequester carbon dioxide through isolation. The deep ocean has a very slow rate of mixing with the surface ocean. Additionally, the surface of the carbon dioxide lake will form a layer of crystal hydrates that will slow dissolution of carbon dioxide into the above ocean. Convective motion over the surface of the lake due to ocean bottom storms or normal sea currents will increase dissolution. Without any bulk flow over the lake, the storage timeline of the carbon dioxide is 10,000 years for a 50 m deep lake. This number is decreased by more than 25 times with currents from ocean bottom storms.[5]
Sites would be chosen based on ocean floor depth, seismic and volcanic activity, and the presence of CaCO3 deposits that could increase the rate of carbon mineralization.[18] Some sites proposed for storage at depths greater than 6 km include the Indonesian Sunda trench, the Japanese Ryukyu trench, and the Puerto Rico trench.[11]
Environmental impacts of deep-sea ocean sequestration
Researchers are studying how ecosystems are affected before and after injection of liquid carbon dioxide through "process studies, surveys of biogeochemical tracers, and ocean bottom studies."[19] The challenge comes from the spatial range of the ocean and the time-scale at which effects would be taking place, making it difficult to detect these effects precisely.[20] There is very limited knowledge as to what organisms and ecosystems exist in this unexplored area and the interdependence of such ecosystems.[20] The following is specifically pertaining to deep ocean sequestration through dilute injection, but touches on alternate methods (injection by towed pipeline, injection by stationary pipeline, use of hydrates). Due to the size of the ocean, the predictions and conclusions regarding the environmental risk of this sequestration process are based on small-scale experiments that have been extrapolated to show possible results on a scale as large as the ocean.[19]
Deep sea biota
Ocean sequestration in deep sea sediments has the potential to impact deep sea life. The chemical and physical composition of the deep sea does not undergo changes in the way that surface waters do.[19] Due to its limited contact with the atmosphere, most organisms have evolved with very little physical and chemical disturbance and exposed to minimal levels of carbon dioxide.[19] Most of their energy is obtained from feeding off of particulate matter that descends from the surface water of the ocean and its ecosystems.[19] Deep sea ecosystems do not have rapid reproduction rates nor give birth to many offspring because of their limited access to oxygen and nutrients.[19] In particular, species that inhabit the 2000-3000m deep range of the ocean have small, diverse populations.[19] Introducing lethal amounts of carbon dioxide into the environment of such a species can have a serious impact on the population size and will take longer to recover relative to surface water species.[21]
Effects of pH vs. carbon dioxide
Acidification of the environment weakens metabolic processes in organisms; enzymes and ion transportation require specific pH levels to continue functioning properly.[19] However, organisms are not only affected by the acidification of water in the presence of heightened carbon dioxide (carbon dioxide) levels. carbon dioxide itself interacts with the physiological function of individual organisms.[19] These effects are more damaging than those associated with changes in pH of environment.[22] When carbon dioxide enters organisms, through diffusion across tissue, it becomes internally accumulated which can then cause anesthesia and, depending on the concentration of carbon dioxide, death.[23] The internal accumulation will also cause organisms to experience blood acidification. This weakens organisms' ability to uptake oxygen and consequently weakens their performances.[19] This effect is more so detrimental to more complex and larger species that require a greater exertion of energy to move and perform vital bodily functions.[19]
Long term effects
If deep-sea ocean sequestration becomes a common practice, long term effects will continue to be investigated to predict future scenarios of deep sea impacts by carbon dioxide.[21] Ocean sequestration of liquid carbon dioxide would not only impact deep-sea ecosystems, but in the long-run would begin to affect surface-water species.[21] It is estimated that organisms not fit for high carbon dioxide levels will begin to experience permanent effects at levels of 400/500ppm of carbon dioxide and/or shifts of 0.1-0.3 units in pH.[19] These levels of carbon dioxide are predicted to be met solely as a result of atmospheric carbon dioxide acidifying the surface waters over a matter of a century, without considering ocean sequestration effects.[19]
Although the long-term effects are the most relevant to understand, they are also the most difficult to predict accurately due to the scale of the ocean and the diversity in species sensitivity to elevated carbon dioxide levels. Surface sea organisms have been better studied than deep-sea animals in terms of consequences due to prolonged carbon dioxide exposure and have been proven to experience "reduced calcification" and damage to their skeletons.[19] This more seriously affects shelled animals' mortality and growth rate.[19] Adult fish showed remarkable tolerance to elevated carbon dioxide levels, only when dissolution of carbon dioxide occurred at a slow rate.[21] Developing fish showed less tolerance than their adult fish counterparts.[21] Acidification of the blood in these species also results in weakened metabolic rates; this stunts protein formation and thus hinders growth and reproduction of organisms.[19] Although individual physiological effects are known, in order to understand how these individual species are interconnected and dependent of each other, field studies would have to be conducted. Different amounts and concentrations of sequestered carbon dioxide will affect each ecosystem and species differently such that a general, universal limit of carbon dioxide to be sequestered doesn't exist.[19]
Corporations in favor of ocean sequestration, such as ExxonMobil,[24] argue that the uncertainties involved with such predictions instill doubt in the conclusions of the research. Supporters of ocean sequestration argue that because of the ocean's size, diluted carbon dioxide injections will not be enough to create an actual impact on ecosystems and that species can evolve to these increased levels of carbon dioxide eventually.[19] Scientific research shows that sites of injection are spatially specific and ecosystems that happen to inhabit the site of injection can suffer immediate consequences.[22] Affected areas will experience acidification, due to the augmented bicarbonate levels, and in turn a decrease in calcium carbonate levels.[22] This will cause sediments and shells of organisms to more quickly dissolve.[19] The capacity of deep-sea organisms to acclimate to the injection of carbon dioxide has not been investigated and the hypothesis that they will evolve in time lacks scientific support.[19]
Minimal impact methods
The use of clathrate hydrates can be implemented in order to reduce speed of dissolution of carbon dioxide.[25][20] The hydrates give carbon dioxide a negative buoyancy, allowing injection to occur at surface levels rather than through pipelines.[21] Experiments showed that the use of clathrate hydrates minimized the rate at which the injected carbon dioxide spread throughout the ocean floor.[26] This rate proved to minimize impact on deep sea organisms.[25] The intactness of the hydrates relies heavily on the ocean current's magnitude at the site of injection.[20] The carbon dioxide dissolved into surface waters before the hydrate was able to sink to the deep ocean (10%-55% of carbon dioxide remained stuck to the hydrate at depths of 1500m into the ocean).[25] In laboratory experiments, continuous streams of hydrates have not yet been achieved.[26]
Studies show that delivering liquid carbon dioxide by a towed pipeline (attached to boat traveling perpendicular to the current), can minimize "clumps" of highly concentrated carbon dioxide levels. Delivery by fixed pipe would be confined to a small region of the ocean and in turn instantly kill sensitive species inhabiting the region. Theoretically, if we assume future anthropogenic emissions of carbon dioxide drop drastically and only 0.37 Gt of liquid carbon dioxide were to be injected each year through a towed pipe, only 1% of the ocean would be affected. There is consensus among scientists that ocean sequestration of carbon dioxide is not a long-term plan to be relied on, but may solve immediate atmospheric concerns if implemented temporarily. Scientists believe that it is possible to engineer ways to discharge carbon dioxide at rates that resemble the natural fluctuation of carbon dioxide in the oceans.[26]
Blue carbon capture in ocean

Ocean storage refers to the use of large water bodies and marine lifeforms to capture carbon by exploiting natural and geological mechanisms. Oceans cover slightly more than 70% of the total surface area of the Earth, and plays a major role in helping to stabilize Earth's climate.[27] This presents itself as a readily available carbon sink to store and capture atmospheric carbon dioxide. Due to the solubility of carbon dioxide in water, CO2 naturally dissolves in oceanic waters to form an equilibrium. With an increase in the concentration of carbon dioxide in the atmosphere, the position of equilibrium pushes the equilibrium in the direction such that more CO2 dissolves into the water. Utilizing this mechanism, more than 500 Gtons of carbon dioxide (amounting to a total of 140 Gtons of carbon) of anthropogenic carbon dioxide emissions released over the past 2 centuries have been absorbed by the oceans.[27] With increasing atmospheric CO2 concentrations released due to human activities as compared to levels before the Industrialization, oceans are currently absorbing 7 Gt carbon dioxide per annum.[14] To enhance the natural mechanism of CO2 dissolving in water, several methods have been proposed by the scientific community. These include the use of iron fertilization, urea fertilization, mixing layers, seaweed,[28][29] as well as direct carbon injection into the sea floor
Iron fertilization
Role of iron in carbon sequestration
Ocean iron fertilization is an example of a geoengineering technique that involves intentional introduction of iron-rich deposits into oceans and is aimed to enhance biological productivity of organisms in ocean waters in order to increase carbon dioxide (CO2) uptake from the atmosphere, possibly resulting in mitigating its global warming effects.[30][31][32][33][34] Iron is a trace element in ocean and its presence is vital for photosynthesis in plants, and in particular phytoplanktons, as It has been shown that iron deficiency can limit ocean productivity and phytoplankton growth.[35] For this reason, “iron hypothesis” was put forward by Martin in late 1980s where he suggested that changes in iron supply in iron-deficient ocean-waters can bloom plankton growth and have a significant effect on the concentrations of atmospheric carbon dioxide by altering rates of carbon sequestration.[36][37] In fact, fertilization is an important process that occurs naturally in the ocean waters. For instance, upwellings of ocean currents can bring nutrient-rich sediments to the surface.[38] Another example is through transfer of iron-rich minerals, dust, and volcanic ash over long distances by rivers, glaciers, or wind.[39][40] Moreover, it has been suggested that whales can transfer iron-rich ocean dust to the surface, where planktons can take it up to grow. It has been shown that reduction in the number of sperm whales in the Southern Ocean has resulted in a 200,000 tonnes/yr decrease in the atmospheric carbon uptake, possibly due to limited phytoplankton growth.[41]
Carbon sequestration by phytoplankton
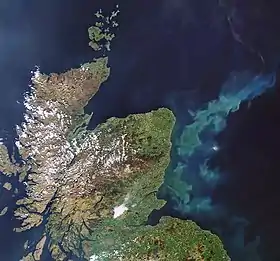
Phytoplankton is photosynthetic: it needs sunlight and nutrients to grow, and takes up carbon dioxide in the process. Plankton can take up and sequester atmospheric carbon through generating calcium or silicon-carbonate skeletons. When these organisms die they sink to the ocean floor where their carbonate skeletons can form a major component of the carbon-rich deep sea precipitation, thousands of meters below plankton blooms, known as marine snow.[42][43][44] Nonetheless, based on the definition, carbon is only considered "sequestered" when it is deposited in the ocean floor where it can be retained for millions of years. However, most of the carbon-rich biomass generated from plankton is generally consumed by other organisms (small fish, zooplankton, etc.)[45][46] and substantial part of rest of the deposits that sink beneath plankton blooms may be re-dissolved in the water and gets transferred to the surface where it eventually returns to the atmosphere, thus, nullifying any possible intended effects regarding carbon sequestration.[47][48][49][50][51] Nevertheless, supporters of the idea of iron fertilization believe that carbon sequestration should be re-defined over much shorter time frames and claim that since the carbon is suspended in the deep ocean it is effectively isolated from the atmosphere for hundreds of years, and thus, carbon can be effectively sequestered.[52]
Efficiency and concerns
Assuming the ideal conditions, the upper estimates for possible effects of iron fertilization in slowing down global warming is about 0.3W/m2 of averaged negative forcing which can offset roughly 15-20% of the current anthropogenic emissions.[53][54][55] However, although this approach could be looked upon as an alternative, easy route, to solving our carbon emission crisis and lower concentration of in the atmosphere, ocean iron fertilization is still quite controversial and highly debated due to possible negative consequences on the marine ecosystem.[48][56][57][58] Research on this area has suggested that fertilization through deposition of large quantities of iron-rich dust into the ocean floor can significantly disrupt ocean's nutrient balance and cause major complications in the food cycle for other marine organisms.[59][60][61][62][63][64][65] Since 1990, 13 major large scale experiments have been carried out to evaluate efficiency and possible consequences of the iron fertilization in ocean waters. A recent research conducted on these experiments determined that the method is unproven; sequestering efficiency is low and sometimes no effect was seen and the amount of iron deposits that is needed to make a small cut in the carbon emissions is in the million tons per year.[66]
Urea fertilization

In waters with sufficient iron micro nutrients, but a deficit of nitrogen, urea fertilization is the better choice for algae growth.[68] Urea is the most used fertilizer in the world, due to its high content of nitrogen, low cost and high reactivity towards water.[22] When exposed to ocean waters, urea is metabolized by phytoplankton via urease enzymes to produce ammonia.[69]
The intermediate product carbamate also reacts with water to produce a total of two ammonia molecules.[23] In 2007 the 'Ocean Nourishment Corporation of Sydney' initiated an experiment in the Sulu sea (southwest of the Philippines), were 1000 tons of urea was injected into the ocean.[22] The goal was to prove that urea fertilization would enrich the algae growth in the ocean, and thereby capture CO2 from the atmosphere. This project was criticized by many institutions, including the European Commission,[70] due to lack of knowledge of side effects on the marine ecosystem.[67] Results from this project are still to be published in literature. Another cause of concern is the sheer amount of urea needed to capture the same amount of carbon as eq. iron fertilization. The nitrogen to iron ratio in a typical algae cell is 16:0.0001, meaning that for every iron atom added to the ocean a substantial larger amount of carbon is captured compared to adding one atom of nitrogen.[5] Scientists also emphasize that adding urea to ocean waters could reduce oxygen content and result in a rise of toxic marine algae.[5] This could potentially have devastating effects on fish populations, which others argue would be benefiting from the urea fertilization (the argument being that fish populations would feed on healthy phytoplankton.[71]
Seaweed fertilization
In order to mitigate global warming, seaweed farming is both a possible and plausible way. This method was adopted in early ocean algae proposals to mitigate global warming. This is done through commercial kelp farms designed to take up tens of thousands of square kilometres of the open ocean.[72] Through this method, seaweed beds will perform as an effective sinks by decreasing the level of dissolved inorganic carbon (DIC) in the ocean.

Seaweeds do the above by removing carbon through the process of photosynthesis, taking in excess CO2 and producing O2. Facts and figures have shown that 0.7 million tonnes of carbon are removed from the sea each year by commercially harvested seaweeds.[73] Even though seaweed biomass is small as compared to the coastal region. They remain essential due to their biotic components, the ability to provide valuable ecosystem services and high primary productivity. Seaweeds are different from mangroves and seagrasses, they are photosynthetic algal organisms[74] and non-flowering. Even so, they are primary producers that grows in the same way as their terrestrial counterparts, both of which assimilate carbon by the process of photosynthesis and generates new biomass by taking up phosphorus, nitrogen, and other minerals.
The attractiveness of large-scale seaweed cultivation is proven over the years, with low-cost technologies and the multiple uses that can be made of its products. Today, seaweed farming made up approximately 25% of the world's aquaculture production and its maximum potential has not been utilised.[75]
Currently in the world, seaweeds contributes approximately 16–18.7% of the total marine-vegetation sink. In 2010 there are 19.2 × tons of aquatic plants worldwide, 6.8 × tons for brown seaweeds; 9.0 × tons for red seaweeds; 0.2 × tons of green seaweeds; and 3.2 × tons of miscellaneous aquatic plants. Seaweed is largely transported from coastal areas to the open and deep ocean, acting as a permanent storage of carbon biomass within marine sediments.[76]
Mixing layers
Mixing layers involve transporting the denser and colder deep ocean water to the surface mixed layer. As the temperature of water in the ocean decreases with depth, more carbon dioxide and other compounds are able to dissolve in the deeper layers.[77] This can be induced by reversing the oceanic carbon cycle through the use of large vertical pipes serving as ocean pumps,[78] or a mixer array.[79] When the nutrient rich deep ocean water is moved to the surface, algae bloom occurs, resulting in a decrease in carbon dioxide due to carbon intake from Phytoplankton and other photosynthetic eukaryotic organisms. The transfer of heat between the layers will also cause seawater from the mixed layer to sink and absorb more carbon dioxide. This method has not gained much traction as algae bloom harms marine ecosystems by blocking sunlight and releasing harmful toxins into the ocean.[80] The sudden increase in carbon dioxide on the surface level will also temporarily decrease the pH of the seawater, impairing the growth of coral reefs. The production of carbonic acid through the dissolution of carbon dioxide in seawater hinders marine biogenic calcification and causes major disruptions to the oceanic food chain.[81]
References
- Marchetti, Cesare (March 1977). "On geoengineering and the CO2 problem" (PDF). Climatic Change. 1 (1): 59–68. Bibcode:1977ClCh....1...59M. doi:10.1007/bf00162777. ISSN 0165-0009. S2CID 153503699.
- Rackley, Stephen A. (2010), "Ocean Storage", Carbon Capture and Storage, Elsevier, pp. 267–286, doi:10.1016/b978-1-85617-636-1.00012-2, ISBN 9781856176361
- "The Earth's Carbon Reservoirs". earthguide.ucsd.edu. Retrieved 2018-12-04.
- Adams, E. Eric, and Ken Caldeira. “Ocean Storage of CO2.” Elements, vol. 4, October 2008, pp. 319–324., doi:10.2113/gselements.4.5.319.
- Caldeira, Ken, et al. “IPCC Special Report on Carbon Dioxide Capture and Storage: Ocean Storage.” International Panel on Climate Change, 2005.
- Paul, Pruess. "Climate Change Scenarios Compel Studies of Ocean Carbon Storage". lbl.gov. Berkeley Lab.
- Herzog, Howard; Caldeira, Ken; Adams, Eric. "Carbon Sequestration via Direct Injection" (PDF). MIT.
- "Ocean Storage of CO2" (PDF). ieaghg.org. IEA Greenhouse Gas R&D Programme.
- ROCHELLE, C. (2003). "CO2 HYDRATE AND UNDERGROUND STORAGE" (PDF). Published Thesis.
- Capron, Mark (July 26, 2013). "Secure Seafloor Storage CO2 Storage" (PDF). Published Thesis.
- Goldthorpe, Steve (2017-07-01). "Potential for Very Deep Ocean Storage of CO2 Without Ocean Acidification: A Discussion Paper". Energy Procedia. 114: 5417–5429. doi:10.1016/j.egypro.2017.03.1686. ISSN 1876-6102.
- House, Kurt (November 10, 2005). "Permanent carbon dioxide storage in deep-sea sediments" (PDF). Proceedings of the National Academy of Sciences. 103 (33): 12291–12295. Bibcode:2006PNAS..10312291H. doi:10.1073/pnas.0605318103. PMC 1567873. PMID 16894174.
- RIDGWELL, ANDY (2007-01-13). "Regulation of atmospheric CO2 by deep-sea sediments in an Earth System Model" (PDF). Global Biogeochemical Cycles. 21 (2): GB2008. Bibcode:2007GBioC..21.2008R. doi:10.1029/2006GB002764.
- https://www.ipcc.ch/pdf/special-reports/srccs/srccs_chapter6.pdf
- Qanbari, Farhad; Pooladi-Darvish, Mehran; Tabatabaie, S. Hamed; Gerami, Shahab (2012-09-01). "CO2 disposal as hydrate in ocean sediments". Journal of Natural Gas Science and Engineering. 8: 139–149. doi:10.1016/j.jngse.2011.10.006. ISSN 1875-5100.
- Zhang, Dongxiao; Teng, Yihua (2018-07-01). "Long-term viability of carbon sequestration in deep-sea sediments". Science Advances. 4 (7): eaao6588. Bibcode:2018SciA....4O6588T. doi:10.1126/sciadv.aao6588. ISSN 2375-2548. PMC 6031374. PMID 29978037.
- Alendal, Guttorm; Drange, Helge (2001-01-15). "Two-phase, near-field modeling of purposefully released CO2in the ocean". Journal of Geophysical Research: Oceans. 106 (C1): 1085–1096. Bibcode:2001JGR...106.1085A. doi:10.1029/1999jc000290. ISSN 0148-0227.
- Bullis, Kevin. "Storing Carbon Dioxide under the Ocean". MIT Technology Review. Retrieved 2018-12-03.
- IPCC, 2005: IPCC Special Report on Carbon Dioxide Capture and Storage. Prepared by Working Group III of the Intergovernmental Panel on Climate Change [Metz, B., O. Davidson, H. C. de Coninck, M. Loos, and L. A. Meyer (eds.)]. Cambridge University Press, Cambridge, United Kingdom and New York, NY, USA, 442 pp.
- Santillo, David., Johnston, Paul. (3 December 2018). "Carbon Capture and Sequestration: Potential Environmental Impacts". CiteSeerX 10.1.1.577.6246. Cite journal requires
|journal=
(help) - Israelsson, Peter H.; Chow, Aaron C.; Eric Adams, E. (2009). "An updated assessment of the acute impacts of ocean carbon sequestration by direct injection". Energy Procedia. 1: 4929–4936. doi:10.1016/j.egypro.2009.02.324.
- Azeem, Babar; KuShaari, KuZilati; Man, Zakaria B.; Basit, Abdul; Thanh, Trinh H. (May 2014). "Review on materials & methods to produce controlled release coated urea fertilizer". Journal of Controlled Release. 181: 11–21. doi:10.1016/j.jconrel.2014.02.020. ISSN 0168-3659. PMID 24593892.
- Kugino, Kenji; Tamaru, Shizuka; Hisatomi, Yuko; Sakaguchi, Tadashi (2016-04-21). "Long-Duration Carbon Dioxide Anesthesia of Fish Using Ultra Fine (Nano-Scale) Bubbles". PLOS ONE. 11 (4): e0153542. Bibcode:2016PLoSO..1153542K. doi:10.1371/journal.pone.0153542. ISSN 1932-6203. PMC 4839645. PMID 27100285.
- Supran, Geoffrey. Oreskes, Naomi "Assessing ExxonMobil's climate change communications" Environmental Research Letters. (1977-2014) (http://iopscience.iop.org/1748-9326/12/8/084019)
- Brewer, Peter G. "Direct Injection of Carbon Dioxide into the Oceans." The Carbon Dioxide Dilemma: Promising Technlogoies and Policies. (2003)
- Israelsson, Peter. Chow, Aaron. Adams, Eric. "An updated assessment of the acute impacts of ocean carbon sequestration by direct injection." Energy Procedia. 2009https://doi.org/10.1016/j.egypro.2009.02.324
- "6. Could CO2 be stored in the deep ocean?".
- De Vooys, 1979; Raven and Falkowski, 1999; Falkowski et al., 2000; Pelejero et al., 2010
- Ortega, Alejandra; Geraldi, N.R.; Alam, I.; Kamau, A.A.; Acinas, S.; Logares, R.; Gasol, J.; Massana, R.; Krause-Jensen, D.; Duarte, C. (2019). "Important contribution of macroalgae to oceanic carbon sequestration". Nature Geoscience. 12 (9): 748–754. doi:10.1038/s41561-019-0421-8. hdl:10754/656768. S2CID 199448971.
- Traufetter, Gerald (2009-01-02). "Cold Carbon Sink: Slowing Global Warming with Antarctic Iron". Spiegel Online. Retrieved 2018-11-18.
- Jin, X.; Gruber, N.; Frenzel, H.; Doney, S. C.; McWilliams, J. C. (2008-03-18). "The impact on atmospheric CO2 of iron fertilization induced changes in the ocean's biological pump". Biogeosciences. 5 (2): 385–406. doi:10.5194/bg-5-385-2008. ISSN 1726-4170.
- "No Title". www-formal.stanford.edu. Retrieved 2018-11-18. Cite uses generic title (help)
- Martínez-García, Alfredo; Sigman, Daniel M.; Ren, Haojia; Anderson, Robert F.; Straub, Marietta; Hodell, David A.; Jaccard, Samuel L.; Eglinton, Timothy I.; Haug, Gerald H. (2014-03-21). "Iron Fertilization of the Subantarctic Ocean During the Last Ice Age". Science. 343 (6177): 1347–1350. Bibcode:2014Sci...343.1347M. doi:10.1126/science.1246848. ISSN 0036-8075. PMID 24653031. S2CID 206552831.
- Pasquier, Benoît; Holzer, Mark (2018-08-16). "Iron fertilization efficiency and the number of past and future regenerations of iron in the ocean". Biogeosciences Discussions. 15 (23): 7177–7203. Bibcode:2018AGUFMGC23G1277P. doi:10.5194/bg-2018-379. ISSN 1726-4170.
- Boyd, Philip W.; Watson, Andrew J.; Law, Cliff S.; Abraham, Edward R.; Trull, Thomas; Murdoch, Rob; Bakker, Dorothee C. E.; Bowie, Andrew R.; Buesseler, K. O. (October 2000). "A mesoscale phytoplankton bloom in the polar Southern Ocean stimulated by iron fertilization". Nature. 407 (6805): 695–702. Bibcode:2000Natur.407..695B. doi:10.1038/35037500. ISSN 0028-0836. PMID 11048709. S2CID 4368261.
- Boyd, P. W.; Jickells, T.; Law, C. S.; Blain, S.; Boyle, E. A.; Buesseler, K. O.; Coale, K. H.; Cullen, J. J.; Baar, H. J. W. de (2007-02-02). "Mesoscale Iron Enrichment Experiments 1993-2005: Synthesis and Future Directions". Science. 315 (5812): 612–617. Bibcode:2007Sci...315..612B. doi:10.1126/science.1131669. ISSN 0036-8075. PMID 17272712. S2CID 2476669.
- "John Martin". earthobservatory.nasa.gov. 2001-07-10. Retrieved 2018-11-19.
- Ian, Salter; Ralf, Schiebel; Patrizia, Ziveri; Aurore, Movellan; S., Lampitt, Richard; A., Wolff, George (2015-02-23). "Carbonate counter pump stimulated by natural iron fertilization in the Southern Ocean". epic.awi.de (in German). Retrieved 2018-11-19.
- (PDF). 2007-11-29 https://web.archive.org/web/20071129123537/http://www.tyndall.ac.uk/events/past_events/ocean_fert.pdf. Archived from the original (PDF) on 2007-11-29. Retrieved 2018-11-19. Missing or empty
|title=
(help) - Hodson, Andy; Nowak, Aga; Sabacka, Marie; Jungblut, Anne; Navarro, Francisco; Pearce, David; Ávila-Jiménez, María Luisa; Convey, Peter; Vieira, Gonçalo (2017-02-15). "Climatically sensitive transfer of iron to maritime Antarctic ecosystems by surface runoff". Nature Communications. 8: 14499. Bibcode:2017NatCo...814499H. doi:10.1038/ncomms14499. ISSN 2041-1723. PMC 5316877. PMID 28198359.
- Lavery, Trish J.; Roudnew, Ben; Gill, Peter; Seymour, Justin; Seuront, Laurent; Johnson, Genevieve; Mitchell, James G.; Smetacek, Victor (2010-11-22). "Iron defecation by sperm whales stimulates carbon export in the Southern Ocean". Proceedings of the Royal Society of London B: Biological Sciences. 277 (1699): 3527–3531. doi:10.1098/rspb.2010.0863. ISSN 0962-8452. PMC 2982231. PMID 20554546.
- J., Brooks; K., Shamberger; B., Roark, E.; K., Miller; A., Baco-Taylor (February 2016). "Seawater Carbonate Chemistry of Deep-sea Coral Beds off the Northwestern Hawaiian Islands". American Geophysical Union, Ocean Sciences Meeting. 2016: AH23A–03. Bibcode:2016AGUOSAH23A..03B.
- Laurenceau-Cornec, Emmanuel C.; Trull, Thomas W.; Davies, Diana M.; Rocha, Christina L. De La; Blain, Stéphane (2015-02-03). "Phytoplankton morphology controls on marine snow sinking velocity". Marine Ecology Progress Series. 520: 35–56. Bibcode:2015MEPS..520...35L. doi:10.3354/meps11116. ISSN 0171-8630.
- Prairie, Jennifer C.; Ziervogel, Kai; Camassa, Roberto; McLaughlin, Richard M.; White, Brian L.; Dewald, Carolin; Arnosti, Carol (2015-10-20). "Delayed settling of marine snow: Effects of density gradient and particle properties and implications for carbon cycling". Marine Chemistry. 175: 28–38. doi:10.1016/j.marchem.2015.04.006. ISSN 0304-4203.
- Steinberg, Deborah K.; Landry, Michael R. (2017-01-03). "Zooplankton and the Ocean Carbon Cycle". Annual Review of Marine Science. 9 (1): 413–444. Bibcode:2017ARMS....9..413S. doi:10.1146/annurev-marine-010814-015924. ISSN 1941-1405. PMID 27814033.
- Cavan, Emma L.; Henson, Stephanie A.; Belcher, Anna; Sanders, Richard (2017-01-12). "Role of zooplankton in determining the efficiency of the biological carbon pump". Biogeosciences. 14 (1): 177–186. Bibcode:2017BGeo...14..177C. doi:10.5194/bg-14-177-2017. ISSN 1726-4189.
- Robinson, J.; Popova, E. E.; Yool, A.; Srokosz, M.; Lampitt, R. S.; Blundell, J. R. (2014-04-11). "How deep is deep enough? Ocean iron fertilization and carbon sequestration in the Southern Ocean" (PDF). Geophysical Research Letters. 41 (7): 2489–2495. Bibcode:2014GeoRL..41.2489R. doi:10.1002/2013gl058799. ISSN 0094-8276.
- Hauck, Judith; Köhler, Peter; Wolf-Gladrow, Dieter; Völker, Christoph (2016). "Iron fertilisation and century-scale effects of open ocean dissolution of olivine in a simulated CO 2 removal experiment". Environmental Research Letters. 11 (2): 024007. Bibcode:2016ERL....11b4007H. doi:10.1088/1748-9326/11/2/024007. ISSN 1748-9326.
- Tremblay, Luc; Caparros, Jocelyne; Leblanc, Karine; Obernosterer, Ingrid (2014). "Origin and fate of particulate and dissolved organic matter in a naturally iron-fertilized region of the Southern Ocean". Biogeosciences. 12 (2).
- Arrhenius, Gustaf; Mojzsis, Stephen; Atkinson, A.; Fielding, S.; Venables, H. J.; Waluda, C. M.; Achterberg, E. P. (2016-10-10). "Zooplankton Gut Passage Mobilizes Lithogenic Iron for Ocean Productivity" (PDF). Current Biology. 26 (19): 2667–2673. doi:10.1016/j.cub.2016.07.058. ISSN 0960-9822. PMID 27641768. S2CID 3970146.
- Vinay, Subhas, Adam (2017). Chemical Controls on the Dissolution Kinetics of Calcite in Seawater (phd). California Institute of Technology. doi:10.7907/z93x84p3.
- Jackson, R. B.; Canadell, J. G.; Fuss, S.; Milne, J.; Nakicenovic, N.; Tavoni, M. (2017). "Focus on negative emissions". Environmental Research Letters. 12 (11): 110201. Bibcode:2017ERL....12k0201J. doi:10.1088/1748-9326/aa94ff. ISSN 1748-9326.
- Lenton, T. M.; Vaughan, N. E. (2009-01-28). "The radiative forcing potential of different climate geoengineering options" (PDF). Atmospheric Chemistry and Physics Discussions. 9 (1): 2559–2608. doi:10.5194/acpd-9-2559-2009. ISSN 1680-7375.
- , "Process and method for the enhancement of sequestering atmospheric carbon through ocean iron fertilization, and method for calculating net carbon capture from said process and method", issued 2016-07-28
- Gattuso, J.-P.; Magnan, A.; Billé, R.; Cheung, W. W. L.; Howes, E. L.; Joos, F.; Allemand, D.; Bopp, L.; Cooley, S. R. (2015-07-03). "Contrasting futures for ocean and society from different anthropogenic CO2 emissions scenarios" (PDF). Science. 349 (6243): aac4722. doi:10.1126/science.aac4722. ISSN 0036-8075. PMID 26138982. S2CID 206639157.
- El-Jendoubi, Hamdi; Vázquez, Saúl; Calatayud, Ángeles; Vavpetič, Primož; Vogel-Mikuš, Katarina; Pelicon, Primoz; Abadía, Javier; Abadía, Anunciación; Morales, Fermín (2014). "The effects of foliar fertilization with iron sulfate in chlorotic leaves are limited to the treated area. A study with peach trees (Prunus persica L. Batsch) grown in the field and sugar beet (Beta vulgaris L.) grown in hydroponics". Frontiers in Plant Science. 5: 2. doi:10.3389/fpls.2014.00002. ISSN 1664-462X. PMC 3895801. PMID 24478782.
- Yoon, Joo-Eun; Yoo, Kyu-Cheul; Macdonald, Alison M.; Yoon, Ho-Il; Park, Ki-Tae; Yang, Eun Jin; Kim, Hyun-Cheol; Lee, Jae Il; Lee, Min Kyung (2018-10-05). "Reviews and syntheses: Ocean iron fertilization experiments – past, present, and future looking to a future Korean Iron Fertilization Experiment in the Southern Ocean (KIFES) project". Biogeosciences. 15 (19): 5847–5889. Bibcode:2018BGeo...15.5847Y. doi:10.5194/bg-15-5847-2018. ISSN 1726-4189.
- Gim, Byeong-Mo; Hong, Seongjin; Lee, Jung-Suk; Kim, Nam-Hyun; Kwon, Eun-Mi; Gil, Joon-Woo; Lim, Hyun-Hwa; Jeon, Eui-Chan; Khim, Jong Seong (2018-10-01). "Potential ecotoxicological effects of elevated bicarbonate ion concentrations on marine organisms". Environmental Pollution. 241: 194–199. doi:10.1016/j.envpol.2018.05.057. ISSN 0269-7491. PMID 29807279.
- Traufetter, Gerald (2009-01-02). "Cold Carbon Sink: Slowing Global Warming with Antarctic Iron". Spiegel Online. Retrieved 2018-11-19.
- "Reuters AlertNet - RPT-FEATURE-Scientists urge caution in ocean-CO2 capture schemes". 2009-08-03. Archived from the original on 2009-08-03. Retrieved 2018-11-19.
- "WWF condemns Planktos Inc. iron-seeding plan in the Galapagos". Geoengineering Monitor. 2007-06-27. Retrieved 2018-11-19.
- "The Global, Complex Phenomena of Harmful Algal Blooms | Oceanography". tos.org. Retrieved 2018-11-19.
- Moore, J.Keith; Doney, Scott C; Glover, David M; Fung, Inez Y (2001). "Iron cycling and nutrient-limitation patterns in surface waters of the World Ocean". Deep Sea Research Part II: Topical Studies in Oceanography. 49 (1–3): 463–507. Bibcode:2001DSRII..49..463M. CiteSeerX 10.1.1.210.1108. doi:10.1016/S0967-0645(01)00109-6. ISSN 0967-0645.
- Trick, Charles G.; Bill, Brian D.; Cochlan, William P.; Wells, Mark L.; Trainer, Vera L.; Pickell, Lisa D. (2010-03-30). "Iron enrichment stimulates toxic diatom production in high-nitrate, low-chlorophyll areas". Proceedings of the National Academy of Sciences. 107 (13): 5887–5892. Bibcode:2010PNAS..107.5887T. doi:10.1073/pnas.0910579107. ISSN 0027-8424. PMC 2851856. PMID 20231473.
- Fripiat, F.; Elskens, M.; Trull, T. W.; Blain, S.; Cavagna, A. -J.; Fernandez, C.; Fonseca-Batista, D.; Planchon, F.; Raimbault, P. (November 2015). "Significant mixed layer nitrification in a natural iron-fertilized bloom of the Southern Ocean". Global Biogeochemical Cycles. 29 (11): 1929–1943. Bibcode:2015GBioC..29.1929F. doi:10.1002/2014gb005051. ISSN 0886-6236.
- Tollefson, Jeff (2017-05-23). "Iron-dumping ocean experiment sparks controversy". Nature. 545 (7655): 393–394. Bibcode:2017Natur.545..393T. doi:10.1038/545393a. ISSN 0028-0836. PMID 28541342. S2CID 4464713.
- Mayo-Ramsay, Julia (September 2010). "Environmental, legal and social implications of ocean urea fertilization: Sulu sea example". Marine Policy. 34 (5): 831–835. doi:10.1016/j.marpol.2010.01.004. ISSN 0308-597X.
- Mingyuan, Glibert, Patricia M. Azanza, Rhodora Burford, Michele Furuya, Ken Abal, Eva Al-Azri, Adnan Al-Yamani, Faiza Andersen, Per Anderson, Donald M. Beardall, John Berg, Gry M. Brand, Larry E. Bronk, Deborah Brookes, Justin Burkholder, JoAnn M. Cembella, Allan D. Cochlan, William P. Collier, Jackie L. Collos, Yves Diaz, Robert Doblin, Martina Drennen, Thomas Dyhrman, Sonya T. Fukuyo, Yasuwo Furnas, Miles Galloway, James Graneli, Edna Ha, Dao Viet Hallegraeff, Gustaaf M. Harrison, John A. Harrison, Paul J. Heil, Cynthia A. Heimann, Kirsten Howarth, Robert W. Jauzein, Cecile Kana, Austin A. Kana, Todd M. Kim, Hakgyoon Kudela, Raphael M. Legrand, Catherine Mallin, Michael Mulholland, Margaret R. Murray, Shauna A. O’Neil, Judith Pitcher, Grant C. Qi, Yuzao Rabalais, Nancy Raine, Robin Seitzinger, Sybil P. Salomon, Paulo S. Solomon, Caroline Stoecker, Diane K. Usup, Gires Wilson, Joanne Yin, Kedong Zhou, Mingjiang Zhu (2008-08-14). Ocean urea fertilization for carbon credits poses high ecological risks. OCLC 1040066339.
- Collins, Carleen M.; D'Orazio, Sarah E. F. (September 1993). "Bacterial ureases: structure, regulation of expression and role in pathogenesis". Molecular Microbiology. 9 (5): 907–913. doi:10.1111/j.1365-2958.1993.tb01220.x. ISSN 0950-382X. PMID 7934918. S2CID 21192428.
- El-Geziry, T M; Bryden, I G (January 2010). "The circulation pattern in the Mediterranean Sea: issues for modeller consideration". Journal of Operational Oceanography. 3 (2): 39–46. doi:10.1080/1755876x.2010.11020116. ISSN 1755-876X. S2CID 130443230.
- Jones, Ian S.F.; Cappelen-Smith, Christian (1999), "Lowring the cost of carbon sequestration by ocean nourishment", Greenhouse Gas Control Technologies 4, Elsevier, pp. 255–259, doi:10.1016/b978-008043018-8/50041-2, ISBN 9780080430188
- "Installing kelp forests/seaweed beds for mitigation and adaptation against global warming: Korean Project Overview". ICES Journal of Marine Science. Retrieved 1 December 2018.
- Israel, Alvaro; Einav, Rachel; Seckbach, Joseph (18 June 2010). "Seaweeds and their role in globally changing environments". ISBN 9789048185696. Retrieved 1 December 2018.
- "Seaweeds: Plants or Algae?". Point Reyes National Seashore Association. Retrieved 1 December 2018.
- "The State Of World Fisheries and Aquaculture" (PDF). Food and Agriculture Organisation of United Nations. Retrieved 1 December 2018.
- Ortega, Alejandra; Geraldi, N.R.; Alam, I.; Kamau, A.A.; Acinas, S.; Logares, R.; Gasol, J.; Massana, R.; Krause-Jensen, D.; Duarte, C. (2019). "Important contribution of macroalgae to oceanic carbon sequestration". Nature Geoscience. 12 (9): 748–754. doi:10.1038/s41561-019-0421-8. hdl:10754/656768. S2CID 199448971.
- "Ocean temperature". Science Learning Hub. Retrieved 2018-11-28.
- Pearce, Fred. "Ocean pumps could counter global warming". New Scientist. Retrieved 2018-11-28.
- Duke, John H. (2008). "A proposal to force vertical mixing of the Pacific Equatorial Undercurrent to create a system of equatorially trapped coupled convection that counteracts global warming" (PDF). Geophysical Research Abstracts.
- US EPA, OW (2013-06-03). "Harmful Algal Blooms | US EPA". US EPA. Retrieved 2018-11-28.
- Shirley, Jolene S. "Discovering the Effects of Carbon Dioxide Levels on Marine Life and Global Climate". soundwaves.usgs.gov. Retrieved 2018-11-28.